It was a balmy day on Earth when animal life on land began. During the Cambrian Period, about 440 million years ago, glaciers had begun to recede, warming the climate and creating new landscapes ripe for sea-dwelling organisms to explore. The creatures that led the charge onto land, though many of their species aren’t known, kicked off the evolutionary tree branch that would lead to the human species.
As species diverged, leading to the animal kingdom, their bodies adapted to their new environments. Yet, in many instances, the genes and biological processes that allowed for survival stayed relatively the same across many species. It is these similarities that allow biomedical researchers to study a variety of organisms to gain insights on human biology.
“Anyone who is studying a biological system is also working on the evolution and history of that biology,” says Gabriel Bever, Ph.D., M.S., an evolutionary biologist at the Johns Hopkins University School of Medicine. “Humans and their diseases are products of these biological processes and histories that have persisted over millions, even billions, of years.”
Bever says the evolutionary tree provides biomedical researchers with a clear progression of biology, which can help them make scientific predictions regarding human physiology and disease. When biomedical researchers study simpler organisms, they are looking at the evolutionary moment in history when humans emerged from these organisms.
“Most of our biology as humans is broadly shared across the evolutionary tree, in flies, worms and even amoebas, who look and behave so differently from us,” says Bever. “But this biology is there because it’s worked for millions, even a billion years, and it’s our job as researchers to question why that is.”
The similarities between humans and their crawly counterparts are essential for efficient biomedical research. If the only creatures that humans are biologically similar to were other humans, model-based research wouldn’t work, limiting the scope of experiments and research in biology. Instead, biomedical researchers can look at simpler model organisms to find insights about human genes, cells and diseases, while working to understand the fundamentals of biology on Earth.
How Amoebas Give Us Hints About Human Cell Structure

In a small petri dish, a tiny, single-celled creature plods onward, lurching forward to a dinner of bacteria. As its slow prowl continues, the organism’s cellular boundaries shapeshift, not only to hold the cell’s inner contents but also to create leg-like projections that help the organism move.
This is Dictyostelium discoideum, a soil-dwelling amoeba that is helping researchers such as Douglas Robinson, Ph.D., uncover insights into the structure of human cells as well as how cancer cells can metastasize and move throughout the body.
Robinson’s journey with amoebas began as a postdoctoral fellow at Stanford University, where he studied and worked on developing tools to observe and manipulate genetic interactions in amoebas. Now, in his lab at the Johns Hopkins University School of Medicine, Robinson uses amoebas to study cell biology, genetics and biophysics.
“I’ve often joked to anyone that asks that humans are really just amoebas with hair,” says Robinson. “Even though their evolution looks different than ours, they’re highly useful in the topics I study.”
In 2006, a team of international scientists sequenced the entire genome of Dictyostelium discoideum amoeba. The creature is relatively simple, with about 13,000 genes. Humans have 20,000–25,000 genes.
About a third of the genes in these amoebas are shared with humans. These “conserved” genes encode for proteins that form structures that are similar between humans and amoebas — this is one reason the single-celled creatures are excellent models to study.
“These organisms are reliable to work with,” says Robinson. “I can determine where to look in the genome, and have easy, accurate ways to observe, and even genetically manipulate, areas of interest in Dictyostelium discoideum.”
Robinson’s primary research focus is how cells are able to change their shape in a variety of scenarios, including cell division or changes to their surrounding environments. His lab studies the network of proteins within a cell that provides structure and shape to the cell, known as the cytoskeleton. Robinson likens the cytoskeleton, which is in both human cells and amoebas, to the structure, or frame, of a house.
Yet, unlike the frame of a house, cytoskeletons are dynamic and moveable, and they can alter the shape of a cell. Different arrangements of the cytoskeleton can give a cell advantages or disadvantages.
Some cytoskeletal positions help a cell divide, whereas others strengthen a cell and help maintain its shape in response to what happens in an amoeba’s environment. It is the adaptability of the cytoskeleton network in human cells that keeps us from becoming blobs of tissue.
Amoebas’ cytoskeletons are similar to the same structure in human cells — it can rearrange to help an amoeba’s movement, feeding or navigation of environmental changes. Robinson can assign a numerical value to these processes in amoeba, with which he and mathematician Pablo Iglesias, Ph.D., create mathematical models that can predict and explain what happens in the amoeba’s system.
These math models help Robinson and his team simulate a biological process, like the shifting of the cytoskeleton as the amoeba moves or engulfs bacteria.
The success or failure of the equations offer researchers hints of whether their interpretations of the amoeba’s processes are accurate. By using mathematical models in tandem with experiments that test the model, Robinson says he can determine and predict the processes in human cells.
“At this point in the research, you’ve asked a foundational question about how biology works using this simple model organism, and now you get to see if it fits in the landscape of humans,” he says.
Using this harmony of math and experiments with amoebas, Robinson’s math model predicted which proteins and parts of the cytoskeleton in a human pancreatic cancer cell would respond to changes caused by a growing tumor in the cell’s environment.
When Robinson’s team studied the pancreatic cancer cell lines, they observed how typically-stationary cells in the pancreas that became cancerous would flee the area and invade other parts of the pancreas and other tissues.
The cytoskeleton’s cellular mechanics that allowed the pancreatic cancer cell to move started with Robinson’s understanding of the cytoskeleton in the amoeba. Knowing how these cancer cells spread in humans means scientists are one step closer to developing better cancer therapies. Indeed, Robinson’s team has already identified a compound that it has tested in animal models of pancreatic cancer, confirming this potential.
Robinson is working on a potential biotech startup based in part on his lab’s research.
“Research that spans across a billion years of evolution is always exciting,” he says. “We always tend to start off thinking about how we differ from creatures like amoebas and worms, but we end up discovering how similar to them we actually are, and how such similarities can help find medical treatments.”
Learning the specifics of human cells with worms
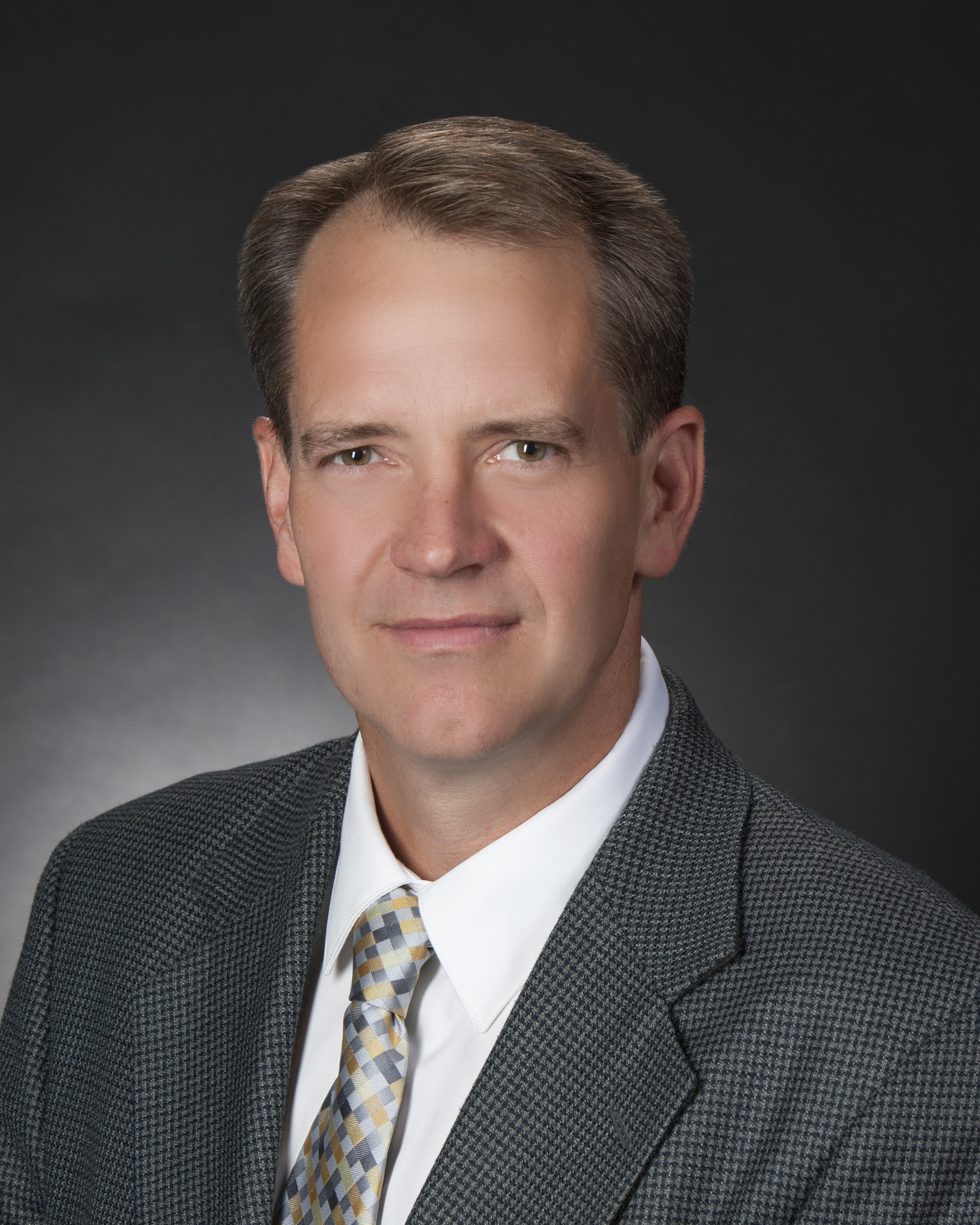
Nematodes, as well as the millions of unicellular and multicellular organisms without bones or hearts, are some of the most successful organisms on the planet. The nematode worm, commonly referred to as C. elegans, has been a successful model organism for research labs around the world since the 1960s.
Researchers have used C. elegans to discover the functions of multiple genes, including the ones responsible for cell death, which are conserved across animals. More than half of the genes from C. elegans have homologs in humans.
It is a favorite model organism of researcher Luisa Cochella, Ph.D., who is working to unravel the mysteries of how genetic programs coax cells into developing into specific cell types, like neurons or muscle cells.
While earning her doctorate at the Johns Hopkins University School of Medicine, Cochella worked next to a research lab that exclusively used C. elegans.
As she conducted experiments of her own, Cochella witnessed how other researchers could precisely influence the genetics and cell biology of C. elegans. When she began to study how cells adopt their final identities, she opted to use C. elegans as a model.
“The true power of worm models in research is their ability to help us uncover principles of biology across species, including giving us insight on clinical aspects of human physiology,” says Cochella. She and her lab use C. elegans to discover new genes and mechanisms, and identify biological processes in the worm’s cells that may be similar to those of human cells.
C. elegans is easy to grow and care for in the lab and it is relatively inexpensive to maintain, but according to Cochella, these worms’ true benefits are their rapid, consistent cell developmental processes and their biological organization.
Each C. elegans, when fully grown, has the same number of cells — precisely 959. This is a manageable number of cells, enabling researchers to quickly see physical changes that occur when they genetically engineer processes in the worm at the molecular level.
From the standpoint of human biology, the cellular and biological functions of C. elegans and the patterns that arise from it’s genetic manipulation are worth studying for clinical reasons. Nearly half of the genes involved in human disease are conserved across both species, meaning that C. elegans has multiple genes with similar functions. Dissecting those gene functions and their physiological consequences is often much more readily done in model organisms such as C. elegans.
Cochella doesn’t specifically study human disease in a C. elegans animal model. Rather, she seeks answers to a fundamental question in biology: How do early embryonic cells know what type of cells to develop into?
To answer this question, Cochella focuses her attention on understanding how genes are turned on or off in specific cell types. An important class of gene regulators is very short, noncoding RNAs known as microRNAs.
MicroRNAs can repress, or turn off, specific genes in a cell during its development. Cochella and her lab have been studying how microRNAs help cells differentiate into muscle cells or neurons.
Because of the biological organization of C. elegans, Cochella has been able to observe microRNA patterns throughout the worm’s entire body, while knowing precisely in which cell each microRNA is expressed.
“One of the new concepts we learned from this was from looking at genes that are needed by every cell to function,” says Cochella. “Surprisingly, we observed that microRNAs that are present in specific cell types targeted some of these universal genes, meaning that even genes that were thought to be universally necessary are tuned in specific cell types, and this helps give them the right functional and morphological properties.”
To study gene-repressing function of microRNAs in different types of C. elegans tissues, Cochella’s lab uses cutting edge genetics, creating nematodes with multiple genetic mutations, sometimes up to six. With this approach Cochella’s team found that when muscle cells lacked a specific microRNA and their target genes couldn’t be repressed, the cells developed abnormal traits that prevented them from functioning as healthy muscle tissue.
Learning how microRNAs, or lack of them, act in multiple types of tissue in the worm has led Cochella to believe that similar processes occur in human cell development, especially in shared disease genes.
“To quote the Nobel laureate Martin Chalfie, these are not just model organisms,” Cochella says. “Rather, they are pioneer organisms, because they’ve driven so much important research. It’s fascinating to me how much we have learned from this microscopic worm.”
Understanding muscle tissue through fruit flies
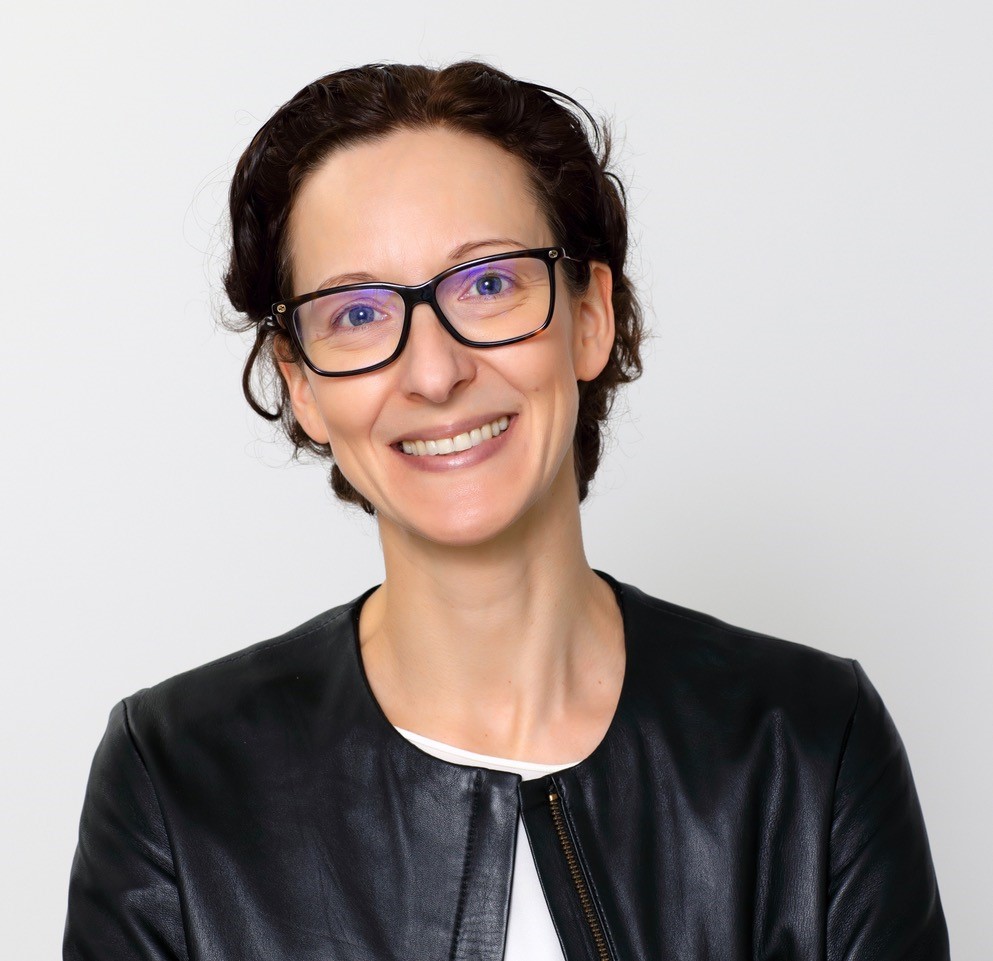
As a fruit fly zips around through the air, potentially annoying you in your kitchen, the muscles that control its wing beats are contracting over 200 times per second, faster than any human muscle can move. Fruit flies are expert fliers, able to travel one meter per second and make 90 degree turns even faster, but being aerial aces isn’t the only thing they can do. In the lab of Johns Hopkins associate professor Anthony Cammarato, Ph.D., fruit flies are helping researchers uncover aspects of proteins that play a role in muscle diseases.
Cammarato first began working with fruit flies — Latin name Drosophila melanogaster — during his graduate studies, when he captured and analyzed zoomed-in photos of individual muscle proteins using an electron microscope.
Over the course of his 10 years at Johns Hopkins, Cammarato has raised and cultivated hundreds of different lines of fruit flies to study a specific type of muscle found both in the insect and in humans.
This kind of muscle is marked by parallel stripes throughout its tissue. Called striated muscle, it is the type that contracts the heart. It also moves the limbs of the body through its attachment to the skeletal system.
In striated muscle, there are two proteins that are key to Cammarato’s work. The first, myosin, acts as a locomotive motor, powering the contraction of muscle fibers. If myosin is the train, the other protein, actin, is the train track. Actin filaments guide myosin movement in order for muscle to contract.
Neither of these proteins can be inserted, expressed and functionally recovered from bacterial cells. However, actin and myosin exist in fruit flies and are similar to the human forms of the proteins.
“We see that the function of these proteins holds across the evolutionary tree, so you can bet that a mutation in the actin of one species is going to have a similar effect on muscle function in another species,” says Cammarato.
He has been able to genetically engineer flies that have the human forms of actin and observe how mutations affect the flies’ muscle physiologies.
Influencing the genetics of a fruit fly is crucial to Cammarato’s work, and the nature of a fly’s genome makes it easy to experiment with. Fruit flies only have four pairs of chromosomes, compared to a human’s 23.
Despite the simplified genome, 70% of fruit fly genes are conserved (have similar versions) in humans. This combination of simple, relevant genetics in addition to the similarity between flies’ and humans’ muscle proteins makes the flies ideal for research on muscle disease and function.
Cammarato has used fruit flies to study the influence of genetic mutations on the striped, striated muscle in the heart. The anatomy of a fly heart is very different than that of human, but it has similar protein constituents and developmental processes.
Rather than a four-chambered organ, the fruit fly heart is a long, linear tube of heart muscle. Also, the fly heart can withstand many mutations, since it isn’t responsible for pumping oxygenated blood to other parts of the fly body.
Many human muscle diseases, like muscular dystrophy or cardiomyopathy, are the result of genetic mutations that involve muscle proteins. Cammarato can manipulate these genes, including those that produce the muscle proteins actin and myosin in the heart, and still observe how the mutations effect the heart muscle in a living fly.
Understanding how heart muscle cells and the muscle proteins actin and myosin react to mutations gives researchers a better idea of possible therapeutic targets for muscle disease.
“The first thing I look for when studying a muscle protein mutation is the evolutionary conservation of the gene or protein across species,” says Cammarato. “If we see similarities in another animal, specifically humans, we can predict the physiological effects of these mutations in diseases.”
During the last six years, Cammarato has also investigated and gained insight specifically on the muscle protein actin, the train track that couples with myosin for muscle contraction.
Using computational chemistry and simulations, Cammarato and his collaborators were able to predict that muscle cells would be unable to properly relax after contracting if a specific region of the actin gene was mutated.
When the researchers mutated this area of the actin gene in flies, their computational predictions came true: Muscles both in the fly heart and striated flight muscles could not properly relax.
Some human muscle diseases, such as certain forms of heart failure, exhibit an inability for muscles to fully and properly relax. Based on these findings, Cammarato believes the flexibility of the actin subunits that form the track is crucial for healthy muscle function, and he says it would have been difficult to reach these conclusions about actin quickly if the researchers had opted to use an animal model other than flies.
“No matter the trends of model organisms in the coming years, I’ll strategically ask questions about muscle biology that can be answered through flies’ unique features,” says Cammarato.
The future of model organisms
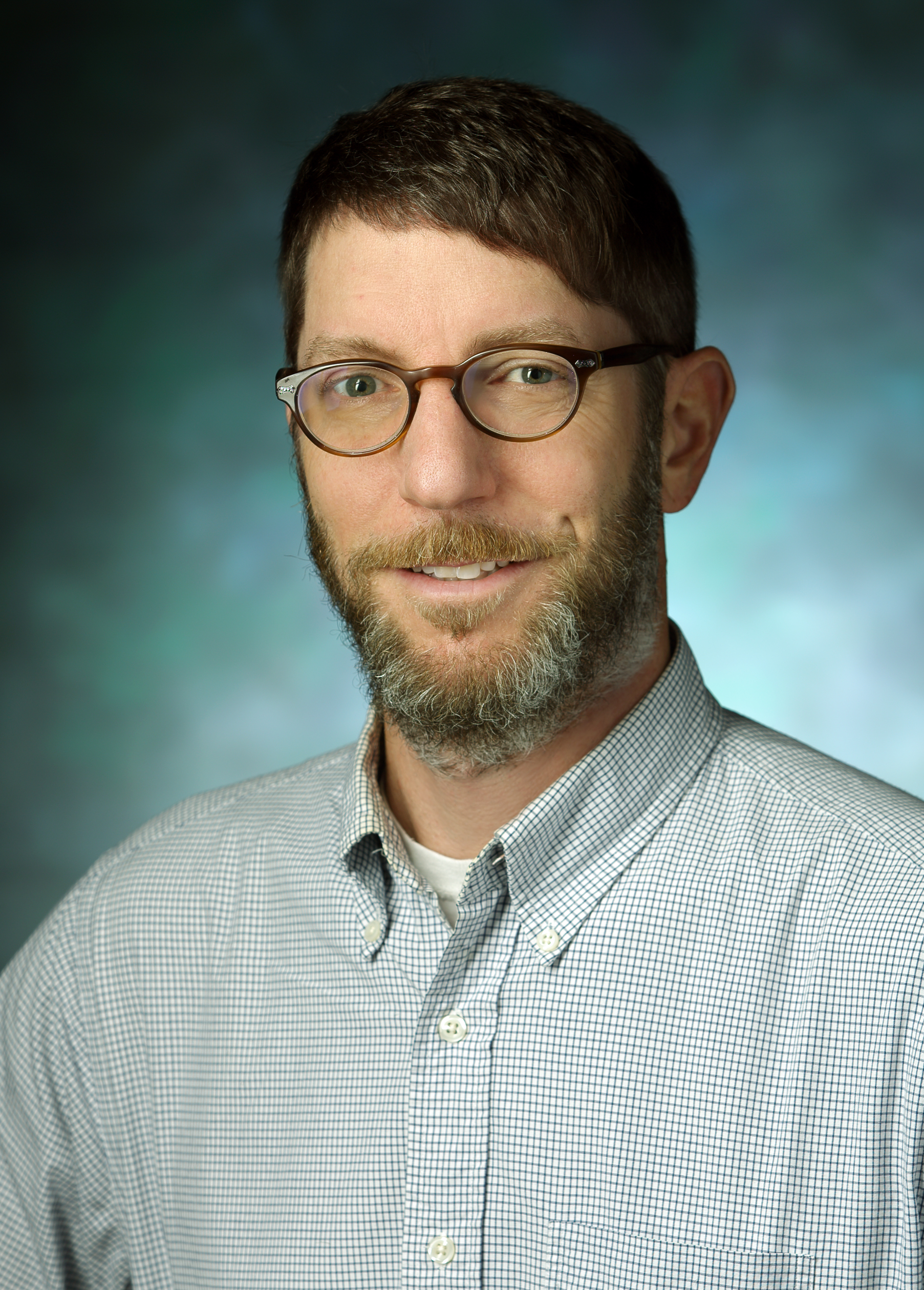
As the hunt for answers in human genetics and disease continues, so will the invaluable biomedical research done with model organisms. More than ever before, biomedical researchers have the scientific tools and methods to analyze the biological processes conserved through evolution and link their findings to aspects of the human body.
“It is such an exciting time to be using model-based approaches in biomedicine,” says Bever. “Somewhere out there, in another species, maybe even in an amoeba, there are solutions to diseases and answers about our workings as humans that we’ll only unlock through these models.”